Harvesting Algae and Other Parts of the Biosphere
This post is a rambling review of a couple of books and a technical paper that are all around a common theme of obtaining renewable resources from photosynthesis.
The books I'll be covering in this post are Harvesting the Biosphere by Vaclav Smil and Slime by Ruth Kassinger. It's my goal to not just provide a summary but to really engage with the content, as the themes are of interest to me on both a personal and professional level.
I read Smil's book at the end of 2019 (as part of this challenge). The first thing I'll say about it is that it is very quantitative. It starts off with a chapter on units and definitions, which is good for ensuring readers are all on the same page, but if you're someone that needs more than a refresher on gigatonnes and terawatts, then it should be taken as fair warning that the whole tome will probably be a slog. Personally, I like that kind of thing; one feature of the book that I appreciated were some boxes that were interspersed with worked-out calculations of different biomass productivities and harvests, starting from clearly explained assumptions. From that starting point, Smil goes through efforts to quantify first the biosphere, then humans' extractions from it. Harvesting the Biosphere covers biomass inventories (stores), productivities (fluxes), harvests of plant and animal biomass, indirect human impacts on the biosphere (through land uses changes, etc.), and the history of foraging and agriculture. Finally, Smil attempts to add up the total human demand on the biosphere, and considers possible future trends.
What does it mean to harvest biomass? Smil talks about "Food, feed, fiber, and wood" (personally I'd go for alliteration as food, feed, fuel, and fibre, since cellulose is a key ingredient in both cotton and lumber) as the key categories of the uses to which people put plants. "Feed" refers to plants used to nourish domesticated livestock; from the big picture view of global ecology taken in Harvesting the Biosphere, eating animals is like eating plants with extra steps. The organic matter that goes to all of these uses originates from organisms that can fix atmospheric inorganic carbon by capturing energy from the sun:
Autotrophs (whose sizes span nearly ten orders of magnitude, from the smallest bacteria at 0.01 μm to the tallest trees at more than 100 m) can transform inorganic carbon (drawn overwhelmingly from atmospheric CO2), hydrogen (mostly from H2O), and smaller amounts of nitrogen and mineral nutrients into complex organic compounds.
Although we put biomass to diverse uses,
more than 90% of biomass is made up of only about 50 different compounds, including 21 amino acids, 15 monosaccharides, 10 fatty acids, and five nucleotides
In most cases, we're interested in properties like the energy content—either as food/feed or fuel (and Smil often reports quantities as an energy equivalent)—the protein content, or structural building blocks (in the case of lumber and fibrous plants). Applications where a specific organic substance (e.g. ivory or natural pigments for dyes) is sought are more niche and generally don't involve large enough quantities to get much focus in a broad book like this.
One of the strengths of Smil's book is that he doesn't just present the overall amounts of biomass inventories, annual yield, or harvested by humans, but dives into where they come from and the limitations/uncertainties of the underlying studies. However, to keep this review to a manageable length, I will jump into the totals without any more preamble, then get into some of the random details I found especially interesting.
The first grand total to consider is the overall inventory of biomass in the world. Obviously this can't be measured directly, so various estimates have been made:
The Pilot Analysis of Global Ecosystems, an attempt to synthesize data from national, regional, and global studies, underscored the continuing uncertainty surrounding phytomass estimations by adopting an excessively broad range of 268–901 Gt C (Matthews et al. 2000), while Saugier, Roy, and Mooney (2001) settled on 652 Gt C and Houghton and Goetz (2008), stressing the continued uncertainties in our understanding of global phytomass, offered a nearly twofold range of 385–650 Gt C.
From his review, Smil thinks a range of 500 - 800 gigatonnes as carbon (midpoint = 650 Gt C) is reasonable. This is the same order of magnitude as carbon in the atmosphere, which is 830 Gt C (at 390 ppm CO2). I wonder if biosphere and atmosphere carbon levels tend to stay in a rough equilibrium?
There are similar uncertainties when it comes to the total annual yield of biomass:
The biosphere’s productivity can be quantified as a cascading series of variables: the most inclusive ones, the two rates at the cascade’s top, gross and net primary productivity, cannot be measured directly and can be quantified (far from accurately) only thanks to our improved understanding of photosynthetic processes, the environmental responses of autotrophs, and the properties of plant metabolism. In contrast, the rates at the cascade’s end can be readily measured in mass or energy terms.
Net primary productivity (NPP) is the one he makes the most use of in Harvesting the Biosphere. Gross primary productivity is the amount of energy captured by photosynthesizers from the sun; NPP is calculated by subtracting their metabolic needs to arrive at the total they can put towards growth. Here are the estimates for NPP:
After two extreme values were excluded, the results ranged from 44.3 to 66.3 Gt C/year with the mean of 54.1 Gt C/year. This translates to roughly 89–132 Gt (mean, 108 Gt) of dry phytomass, or, assuming an average energy density close to 20 GJ/t, to about 2 ZJ. Two conceptually similar models of terrestrial and marine NPP resulted in a combined global total of 104.9 Gt C/year, with 56.4 Gt C/year on land and 48.5 Gt C/year in the ocean (Field et al. 1998; Geider et al. 2001). The most likely planetary total of satellite-based modeling of NPP was thus between 100 and 110 Gt C/year or 200–220 Gt/year of dry phytomass and at least 3.5 ZJ of energy (an annual flux of about 110 TW).
Taking the middle of the ranges stated gives an estimated global NPP of 105 gigatonnes per year as carbon, split almost equally between the land and water (54 Gt C/yr and 51 Gt C/yr, respectively). However, the surface area of water is 2.4 times higher (36 vs 15 gigahectares), so the productivity on land is higher. I'll discuss this more below. Comparing the biomass stores and flux yields an average turn-over time of around 6 years. Smil uses some rough equivalence factors—which is all that can be done in such a zoomed-out analysis—to estimate the dry weight of phytomass to be double the mass as carbon (the live/wet weight will be higher still, but water content varies widely) and the energy content to be 20 gigajoules per dry tonne. I did some calculations on cellulose (C6H10O5, the most abundant biopolymer) for comparison to these factors: it is 44% carbon by mass, with an energy density of 17 GJ/t.
The capture of solar energy is calculated as 1.1 x 1014 watts. Solar irradiance is around 1 kW per m2 but once night and clouds are accounted for, it's more like 200 W/m2 (see this image for reference); multiplying by a global surface area of 5.1 x 1014 m2 indicates 0.1% of incoming solar energy is converted into biomass. This fraction is a consequence of the efficiency of photosynthesis and global coverage of plants and other photosynthesizers. Going to higher trophic levels (herbivores then carnivores), only around 10% of energy consumed can be converted into new biomass. To put the amount of energy involved in further context, the US uses around 0.1 ZJ (100 quadrillion BTU) annually, compared to the 3.5 ZJ Smil states.
The theme of this book, of course, is not just the biosphere but also how much of its productivity is captured by humanity. Out of NPP, significant amounts of phytomass are consumed by animals or fungi, or comprise roots and other parts of plants that we don't find readily usable. And some phytomass is used as feed for livestock rather than directly as food, with the energy conversion losses that implies. There are different ways of looking at the amount of biosphere productivity that humans end up claiming. All of them come with the caveat that,
the biosphere operates in ways that make it impossible for humans to take exclusive possession of any phytomass.
Different values from different studies are presented in the book. One of them is:
During the first decade of the twenty-first century the annual harvest (and direct destruction) of terrestrial phytomass thus added up to roughly 20 Gt of dry matter, or about 10 Gt C; this translates to nearly 17% of the biosphere’s annual terrestrial NPP
In another place Smil states that crops consumed by people or our livestock (plus the residues from processing them) add up to around 2.6 Gt C/yr. Phytomass is also consumed for energy, land clearance, and by wildfires started by people. Another way of looking at humanity's claim on the biosphere is the footprint of agriculture:
by 2010 the land used for annual and permanent crops had surpassed 1.5 Gha, claiming about 12% of all ice-free land, but the peak seasonal preharvest phytomass of global cropland is less than 0.5% of all plant mass.
The totals above encompass a lot of variability. When it comes to the stores of phytomass in different biomes, temperate grasslands have 10-20 dry tonnes per hectare, wetlands have 20-60, and boreal forests have 40-100. The timeframe in which this biomass undergoes a turn-over also varies widely. Peak net annual productivity (in the Amazon and Southeast Asia) is around 10 tonnes per hectare as carbon (around 20 tonnes per hectare dry weight and 400 GJ/ha using Smil's back-of-the-envelope conversions). The poles, deserts, and nutrient-poor areas of the open ocean will be far less, of course.
Having reviewed the big picture view that is the core of Harvesting the Biosphere, I want to share some other things it covered that I found interesting, including biomass used for energy or grazing.
Brazilian sugarcane has the highest yield of any crop, at 75 tonnes/hectare (fresh weight, of which around 12% is sucrose). The output from a hectare can be converted into around 5,000 litres of ethanol. Corn yields have increased dramatically over the past century but are not as high (10 tonnes per hectare) and thus the ethanol output per hectare is also lower.
In previous times (and still in some places), woody biomass was a major source of energy. One of the calculation boxes I mentioned above goes into the amount of charcoal that would have been consumed by a blast furnace in the eighteenth century for smelting pig iron. A single furnace could go through 12,000 tonnes of wood to smelt 300 tonnes of metal. Although efficiencies have improved since then, it's very good for the world's forests that non-biomass sources of energy have been found:
These realities should be kept in mind by all advocates of green energy futures. Blast furnaces produced about 50 Mt of iron in 1900, 580 Mt in 2000, and nearly 1,000 Mt (1 Gt) in 2010. By 1910 the average worldwide ratio of dry coke to hot metal had declined to just 0.45:1, which means that the global need for pig iron smelting was about 450 Mt of coke. Replacing all of this with charcoal would require—even when assuming just 0.7 t of charcoal per 1 t of hot metal and a 3.5:1 wood: charcoal ratio—about 2.5 Gt of wood, the total roughly equal (assuming average 650 kg/m3) to the world’s harvest of about 3.9 Gm3 of roundwood. And even if that wood were to come from high-yielding tropical eucalyptus (producing annually 10 t/ha), its growth would occupy some 250 Mha, an equivalent of more than half of Brazil’s Amazon forest.
And that's just the energy demand of smelting. Another calculation box considers the amount of wood that went into large sailing ships at their zenith (4,000 tonnes, plus 2,000 tonnes trimmed off in the construction process) and states that it could have provided the annual heating needs of 1,500 households. When wood was the main source for household and industrial energy needs in addition to being the primary building material, it was consumed at a prodigious rate. From this historical perspective, Smil is not highly enthusiastic about biofuels being a sustainable source of energy. He also points out that even cellulosic ethanol, which would use crop residues rather than food crops, would still be competing for phytomass that currently serves for animal fodder or helps maintain and regenerate soil.
One of the conclusions I drew from Harvesting the Biosphere is that in Smil's judgement, it's better not to rely on the biosphere for our energy needs (so a mix of nuclear power and renewables would probably be the most viable route to reduce dependency on fossil fuels). Hopefully it can continue to be enough for our other needs of food, feed, and fibre.
Some of the statistics and discussion around grazing were interesting. Smil reports that in Masai Mara National Park in Kenya the live weight of large wild mammals works out to 100 kg/ha. A calculation box about grazing cattle (also in East Africa) has densities that work out to 25 kg/ha (around 10 hectares per cow), with each cow consuming 1.5 dry tonnes of grass per year. But grazing shouldn't be seen as just taking from the ecosystem. Grazers (wild and domestic) fertilize grasslands and so contribute to its productivity. Smil writes that,
Modern appraisals see a properly practiced pastoralism not as an archaic way of food production that is conducive to land degradation and can provides only negligible economic benefits but as a rational, environmentally appropriate, and potentially rewarding option to exploit the limited primary productivity of arid ecosystems
Compared to even the most teeming natural ecosystems, the population density of cities is astounding. I'll use a different example than Smil, but he makes the same point. Vancouver is the densest city in Canada (NYC is twice as dense), with 5500 people per square kilometer (probably over 2000 kg/ha). A 2000 Calorie per day diet adds up to 3 GJ/yr, so even the most productive ecosystems can only support 100 - 150 people per hectare—if their food needs are all met from plants, the net primary productivity is fully captured and not shared with other heterotrophs, and non-food needs (fuel, fibre, etc.) are negligible. A lot of ancient civilizations were only at 1% of this density or slightly more. The fact that modern cities can exceed this density by so much is a testament to the far flung hinterlands they effectively rely on, and to the connective logistics.
Most of this review up to now has focused on the terrestrial biosphere, just as the book does. But it does have a bit to say about the marine biosphere. One of its key features is that most biomass in the oceans is heterotrophs:
The situation is reversed in the ocean as phytoplankton’s short life span limits the total amount of standing autotrophic biomass[*] and results in inverted trophic pyramids, with the aggregate heterotrophic biomass being commonly twice, and up to four times, as large as the biomass of primary producers and with some fish and marine mammals being fairly massive.
*Elsewhere he provides a citation that phytoplankton has a standing mass of only 1 - 4 gigatonnes as carbon, and a turnover time of 2-6 days. This would seem to imply a higher NPP than the ~50 Gt C/yr quoted above.
Shares of the NPP that are actually consumed by herbivores are only 1%–2% in deciduous temperate forests, may surpass 25% in temperate meadows and wetlands, and reach maxima of 50%–60% in rich tropical grasslands; in the ocean they can peak at more than 95% in some patches of phytoplankton
This means that most of what humanity harvests from the sea is animals (fish, shellfish, etc.). Harvesting higher trophic levels has a multiplier effect in terms of the burden on the primary productivity of the ocean, so overfishing is a concern. I was reminded of a documentary I watched that referred to "the rise of slime", where diminished numbers of fish to graze on them leads to an unhealthy proliferation of phytoplankton and invertebrates (another episode of the same documentary talked about the benefits of grazing, as referred to above).
Still, I think the oceans have a lot of untapped potential, if stewarded in the way on-land agriculture has been to increase yields (and here's an article on how even on-land production of animal feed could be significantly further intensified) and diversifying harvests away from apex species. Seasteading discussed ways to enhance oceanic productivity by inducing upwelling of nutrient-rich water from the seafloor to the sunlit surface, to list one possibility.
This discussion of tapping more into marine productivity is a good spot for moving on to Ruth Kassinger's book. I'll start where she does, with a definition of algae:
So, what exactly are algae? There is no exact answer. Algae is not a precise term, not a taxonomic category like the kingdom Animalia or the genus Homo or the species Homo sapiens. Algae (and the singular alga) is a catchall term, a name for a group of diverse organisms. There are three types, ... blue-green algae, now generally known as cyanobacteria ... microalgae ... [and] macroalgae.
Cyanobacteria and microalgae are both single-celled phytoplankton, while macroalgae are multicellular and colloquially known as seaweed. The description continues with some characteristics that make the relevance of algae to this post clear:
You will never find algae dressed in flowers, wafting scents, or sporting seeds and berries. Plants are the fancy-pants photosynthesizers of our world; algae are the plain Janes. But because algae have no petals or nectar, no pistils or stamens, no bark to keep them from drying out, and no wood to hold them upright, they channel far more of the sun’s energy into multiplying themselves. And that means they are dozens of times more productive than plants at making the carbohydrates, proteins, vitamins, and oils—as well as accumulating the minerals—that we value. [emphasis added]
Slime is divided into four sections. The first section was like a textbook excerpt, summarizing the current scientific understanding about algae. I found the book got a lot more interesting in subsequent sections where the author started discussing how it has been and continues to be harvested for human use. These sections used a style of writing that I almost always enjoy, where the writer takes readers along on their research journey by describing the places they go and the people they talk to, rather than just sharing their findings. Kassinger certainly went a lot of places: both sides of the Gulf of Maine, South Korea, the British Isles, the southern US. She covers topics such as algae as food products in various cultures; modern cultivation efforts as animal feed, fertilizer, biopolymers, and fuel; and finally environmental issues related to algae. The environmental issues span from negative impacts such as algae blooms and coral bleaching, to possible solutions like water treatment (see below) and even geoengineering. At the back of the book there is an appendix with some suggested recipes for cooking with algae.
For my review, I'll focus on the industrial biotechnology stories that Kassinger discusses, as that's an area I have a professional interest in. Although algae have had some industrial uses for a long time—she mentions people in the Orkney Islands going kelping then burning the seaweed to make ash (soda ash and potash; 20,000 tons of ash from 400,000 tons of seaweed) for glassmaking two hundred years ago—it has seen a surge of interest lately. Like any field brimming with optimism, not every venture has been a success. I appreciated the clear-eyed coverage of the sector. The saga of Sapphire Energy stands out as an example:
Of all the companies in the burgeoning sector, Sapphire Energy gathered the most press coverage and funding. In early 2008, the company was working out of subleased space with just three offices, a couple of lab benches, and an unused greenhouse, but it soon secured more than $100 million in capital from Bill Gates’s investment firm, Cascade Investment, as well as Venrock, Arch Venture, and the Wellcome Trust.
"But it’s impossible for any alternative fuel to compete at $30 a barrel.” In 2015, Sapphire closed down its algae fuel operations. From a peak of 140 employees, Sapphire had retained about 40 in February of 2016 when I visited. Like many former algae fuel companies, the company had by then recognized that its survival depended on producing higher-value, lower-volume products. When I spoke with Behnke and Yohn, they were trying to pivot to pigments, proteins, nutraceuticals, and fish and animal feed.
Algenol is another company that attempted to make biofuel (ethanol in this case) from algae:
Algenol’s microbiologists have inserted certain yeast genes into cyanobacteria’s genome, which enable them to ferment the sugars they make via photosynthesis and trickle out ethanol.
By 2010, Algenol was producing ethanol on a demonstration scale at a rate of more than 2,500 gallons per year per acre [(24,000 L/ha/yr)] of barren scrub in Florida—six times the amount of ethanol a Kansas corn farmer could produce on an acre of farmland. That was impressive, but Woods had calculated that it would take more than six thousand gallons per acre to reach his goal of selling ethanol, without subsidies, for under $1.30 per gallon.
It takes one acre of well-fertilized, extensively watered, arable land to produce about four hundred gallons of corn ethanol per year [(3,800 L/ha/yr)]. Algenol uses just one acre of nonarable land, no fresh water, minor amounts of fertilizers, and potentially free carbon dioxide to produce, according to Woods, about nine thousand gallons of algae ethanol. Instead of turning fresh water and corn into fuel, it turns saltwater and a greenhouse gas into fuel, with fertilizer and fresh water left over.
While they still appear to be around, their website now makes no mention of fuel and instead offers niche products that command a higher margin. As Smil discussed, biofuels are—at least for the present—very difficult to make the numbers pencil out for stand-alone production. The potential upsides mean people will surely continue to try, but anyone who does would be well advised to be able to pivot to a premium product as a back-up plan, as that's what the companies that have survived seem to have done.
Solazyme (later known as TerraVia) is another company covered in the book. They have gone bankrupt in recent years but their assets were purchased by Corbion, so production of at least some of their products continues. Another very unique product made from algae covered in this book is the renewable plastic (ethylene vinyl acetate) made by Algix and used to make sneakers (in the paper I discuss below they consider sending algae to Algix as a feedstock for bioplastics).
I feel a slight connection to one of the stories covered in Slime, partly because the company is headquartered in the region where I live, and partly because the company I work for supplied a wastewater treatment system for a carrageenan processing plant in the Philippines.
The expansion was fortunate; by the early 1990s, Acadian’s Irish moss business was melting away. The cottonii farmers in East Asia were supplying carrageenan-rich seaweed at prices no one in North America could match. Acadian exited[*] the carrageenan business, leaving it to the Philippines and Indonesia. Today, the carrageenan business is a mainstay of coastal villages in the Philippines, where small-scale family operations grow the bulk of the seaweed. In the twenty-first century, carrageenan farming has helped save tens of thousands of people from poverty.
*Their current seaweed products include plant growth stimulants and animal feed
Speaking of water treatment, Kassinger mentions a floway near Vero Beach, Florida that uses algae to absorb nutrients from stormwater:
Over the course of 2016, this floway will produce almost seven million pounds of wet algae. It’s only our first year at Osprey Marsh, but based on the data so far, that means we’ll be taking about five thousand pounds of phosphorus and fifteen thousand pounds of nitrogen out of the water. That’s twenty thousand pounds of nutrients that never enter the lagoon.
While that's certainly not a trivial amount, it only works out to 10 pounds of nitrogen per acre per day (11.5 kg/ha/d). The overall yield is 2000 tonnes/ha/yr of fresh weight, more than 25 times the yield for sugarcane that Smil cites (although the water content is probably significantly higher, so I wish dry weights were given for comparison).
While I haven't yet tried any of the recipes included with the book (or from a website the author recommended; and here's another online guide to cooking with seaweed), Slime did inspire some scones my fiancée made using dulse as one of the ingredients. They were very tasty.
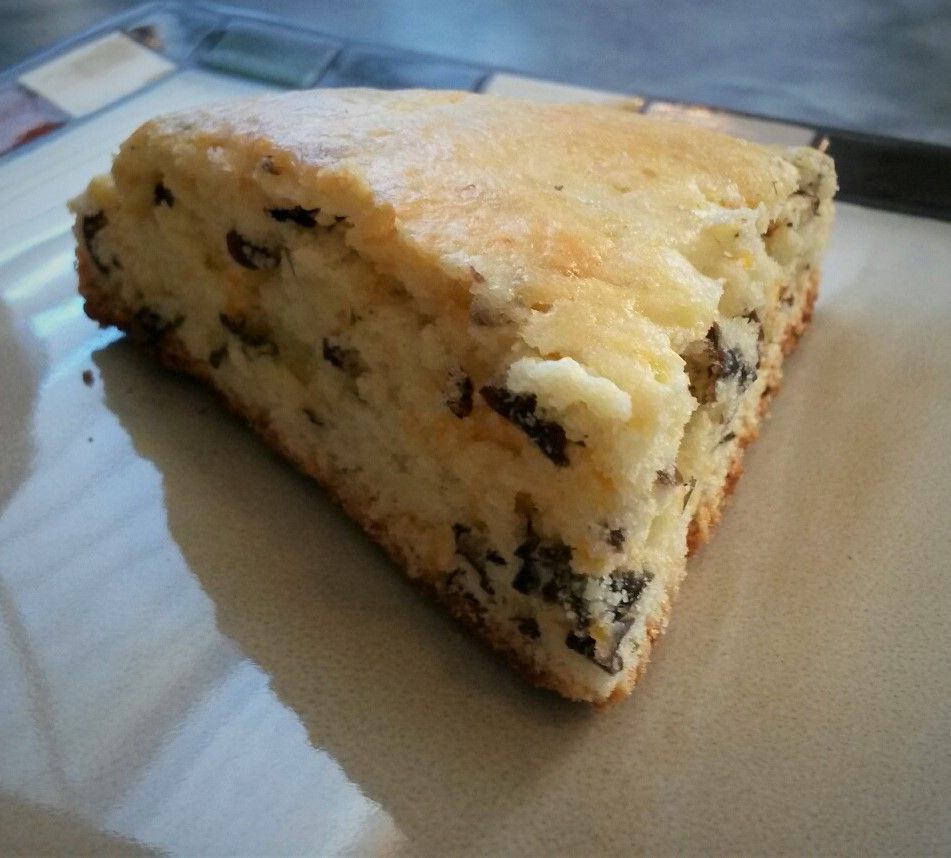
Finally, I want to briefly discuss a paper I read on using algae for wastewater treatment. "The potential of nutrient recovery from wastewater using a revolving algae biofilm system and beneficial utilization of algae biomass" by Kumar et al. (2019) was presented at the WEFTEC conference. Some of the authors are affiliated with Gross-Wen Technologies, which has a "revolving algal biofilm" (RAB) technology that the study is based on. The RAB comprises belts for algae to grow on that are partially submerged in water. As they rotate, the algal biofilm is alternately exposed to water, where it can absorb dissolved nutrients and keep from drying out, and air, where it can obtain CO2 (an important nutrient for autotrophs) and receive more light. Part of the study was to attempt to find the right balance between the time in the water and in the air. The belt arrangement also provides more surface area for growth compared to a raceway, reducing the overall footprint of the system.
Part of the motivation for a system like this is to recover phosphorus in a usable form:
Since P is a non-renewable resource required for all life on this planet, meeting anticipated P limits by means of recovering the P as a resource, which can then be reintroduced to the terrestrial cycle, is a more sustainable method than traditional P-removal approaches such as chemical precipitation.
They were able to achieve decent removals of nutrients from secondary municipal effluent:
Preliminary results [for a 3.05 m tall RAB] at steady-state conditions showed ortho-P and ammonia removal efficiencies of 80% within a 1.4-day HRT.
For comparison with the books discussed in this post, the productivity of the algal biomass is a good thing to consider. Figure 5 in the paper, based on some modelling they did, shows 3.5 g/m2/d (based on the belt surface area) or 22 g/m2/d (based on the footprint). They are equivalent to 12.8 tonnes/ha/yr and 80 tonnes/ha/yr, respectively (if I understand correctly, these are dry weights); they claim adding supplemental lighting can increase productivity by a factor of ~3.
The midpoints of the nutrient content of the algal biomass were 6.3% and 1.8%, for nitrogen and phosphorus, respectively. For comparison this paper (in table 3) has a similar nitrogen content of 5.1 - 6.3% in different types of algae (and an energy value of 18 - 20.5 MJ/kg, which lines up well with factors Smil used). Combining the nutrient content with the biomass productivity gives a result that a single unit (150 m2 surface area; and assuming no supplemental lighting) could remove around 9 g/d of phosphorus. So the footprint for a full-scale application would be very large.
To wrap up this post, I'll share a photo of an exhibit that Micropia (a museum in Amsterdam) had about algae and things that can be made from them: